Imagine you are a young Bar-tailed Godwit, a large, leggy shorebird with a long, probing bill hatched on the tundra of Alaska. As the days become shorter and the icy winter looms, you feel the urge to embark on one of the most impressive migrations on Earth: a nonstop transequatorial flight lasting at least seven days and nights across the Pacific Ocean to New Zealand 12,000 kilometers away. It’s do or die. Every year tens of thousands of Bar-tailed Godwits complete this journey successfully. Billions of other young birds, including warblers and flycatchers, terns and sandpipers, set out on similarly spectacular and dangerous migrations every spring, skillfully navigating the night skies without any help from more experienced birds.
People have long puzzled over the seasonal appearances and disappearances of birds. Aristotle thought that some birds such as swallows hibernated in the colder months and that others transformed into different species—redstarts turned into robins for the winter, he proposed. Only in the past century or so, with the advent of bird banding, satellite tracking and more widespread field studies, have researchers been able to connect bird populations that winter in one area and nest in another and show that some travel vast distances between the two locales every year. Remarkably, even juvenile long-haul travelers know where to go, and birds often take the same routes year after year. How do they find their way?
Migrating birds use celestial cues to navigate, much as sailors of yore used the sun and stars to guide them. But unlike humans, birds also detect the magnetic field generated by Earth’s molten core and use it to determine their position and direction. Despite more than 50 years of research into magnetoreception in birds, scientists have been unable to work out exactly how they use this information to stay on course. Recently we and others have made inroads into this enduring mystery. Our experimental evidence suggests something extraordinary: a bird’s compass relies on subtle, fundamentally quantum effects in short-lived molecular fragments, known as radical pairs, formed photochemically in its eyes. That is, the creatures appear to be able to “see” Earth’s magnetic field lines and use that information to chart a course between their breeding and wintering grounds.
A Mysterious Sense
Migratory birds have an internal clock with an annual rhythm that tells them, among other things, when to migrate. They also inherit from their parents the directions in which they need to fly in the autumn and spring, and if the parents each have different genetically encoded directions, their offspring will end up with an intermediate direction. For example, if a southwest-migrating bird is crossed with a southeast-migrating bird, their offspring will head south when the time comes. But how do the young birds know which direction is southwest or south or southeast? They have at least three different compasses at their disposal: one allows them to extract information from the position of the sun in the sky, another uses the patterns of the stars at night, and the third is based on Earth’s ever present magnetic field.
In their first autumn, young birds follow inherited instructions such as “fly southwest for three weeks and then south-southeast for two weeks.” If they make a mistake or are blown off course, they are generally unable to recover because they do not yet have a functioning map that would tell them where they are. This is one of the reasons why only 30 percent of small songbirds survive their first migrations to their wintering grounds and back again. During its first migration a bird builds up a map in its brain that, on subsequent journeys, will enable it to navigate with an ultimate precision of centimeters over thousands of kilometers. Some birds breed in the same nest box and sleep on the same perch in their wintering range year after year. Equipped with this map, about 50 percent of adult songbirds make it back to their nesting site to breed every year.
Migratory birds’ navigational input comes from several senses—mainly sight, smell and magnetoreception. By observing the apparent nighttime rotation of the stars around the North Star, the birds learn to locate north before they embark on their first migration, and an internal 24-hour clock allows them to calibrate their sun compass. Characteristic smells can help birds recognize places they have visited before. Scientists know a great deal about the detailed biophysical mechanisms of the birds’ senses of sight and smell. But the inner workings of their magnetic compass have proved harder to understand.
The magnetic direction sense in small songbirds that migrate at night is remarkable in several important respects. First, observations of caged birds exposed to carefully controlled magnetic fields show that their compass does not behave like the magnetized needle in a ship’s compass. A bird detects the axis of the magnetic field and the angle it makes with Earth’s surface, the so-called inclination compass. In laboratory experiments, inverting the magnetic field’s direction so that it points in exactly the opposite direction has no effect on the bird’s ability to orient correctly. Second, a bird’s perception of Earth’s magnetic field can be disrupted by extraordinarily weak magnetic fields that reverse their direction several million times per second. Last, even though songbirds fly at night under the dim light of the stars, their magnetic compass is light-dependent, hinting at a link between vision and magnetic sensing.
In 1978, in an attempt to make sense of these features of avian magnetoreception, Klaus Schulten, then at the Max Planck Institute for Biophysical Chemistry in Göttingen, Germany, put forth a remarkable idea: that the compass relies on magnetically sensitive chemical transformations. At first glance, this proposal seems preposterous because the energy available from Earth’s magnetic field is millions of times too small to break, or even significantly weaken, the bonds between atoms in molecules. But Schulten was inspired by the discovery 10 years previously that short-lived chemical intermediates known as radical pairs have unique properties that make their chemistry sensitive to feeble magnetic interactions. Over the past 40 years researchers have conducted hundreds of lab studies of radical-pair reactions that are affected by the application of magnetic fields.
To appreciate why radical pairs are so special, we need to talk about a quantum-mechanical property of the electron known as spin angular momentum, or “spin” for short. Spin is a vector with a direction as well as a magnitude, and it is often represented by an arrow, ↑ or ↓, for example. Particles with spin have magnetic moments, which is to say they behave like microscopic magnets. Most molecules have an even number of electrons arranged in pairs with opposed spins (⇅), which therefore cancel each other out. Radicals are molecules that have lost or gained an electron, meaning that they contain an odd, unpaired, electron and hence have a spin and a magnetic moment. When two radicals are created simultaneously by a chemical reaction (this is what we mean by radical pair), the two unpaired electrons, one in each radical, can have either antiparallel spins (⇅) or parallel spins (↑↑), arrangements known as singlet and triplet states, respectively.
Immediately after a radical pair is created in a singlet state, internal magnetic fields cause the two electronic spins to undergo a complex quantum “waltz” in which singlet turns into triplet and triplet turns back into singlet millions of times per second for periods of up to a few microseconds. Crucially, under the right conditions, this dance can be influenced by external magnetic fields. Schulten suggested that this subtle quantum effect could form the basis of a magnetic compass sense that might respond to environmental stimuli a million times weaker than would normally be thought possible. Research that we and others have carried out in recent years has generated fresh support for this hypothesis.
.png)
A Possible Mechanism
To be useful, hypotheses need to explain known facts and make testable predictions. Two aspects of Schulten’s proposed compass mechanism are consistent with what is known about the birds’ compass: radical pairs are indifferent to exact external magnetic field reversals, and radical pairs are often formed when molecules absorb light. Given that the birds’ magnetic compass is light-dependent, a prediction of Schulten’s hypothesis is that their eyes play a part in the magnetic sensory system. About 10 years ago the research group of one of us (Mouritsen) at the University of Oldenburg in Germany found that a brain region called Cluster N, which receives and processes visual information, is by far the most active part of the brain when certain night-migrating birds are using their magnetic compass. If Cluster N is dysfunctional, research in migratory European Robins showed, the birds can still use their sun and star compasses, but they are incapable of orienting using Earth’s magnetic field. From experiments such as these, it is clear that the magnetic compass sensors are located in the birds’ retinas.
One early objection to the radical-pair hypothesis was that no one had ever shown that magnetic fields as tiny as Earth’s, which are 10 to 100 times weaker than a fridge magnet, could affect a chemical reaction. To address this point, Christiane Timmel of the University of Oxford and her colleagues chose a molecule chemically unlike anything one would find inside a bird: one that contained an electron donor molecule linked to an electron acceptor molecule via a molecular bridge. Exposing the molecules to green light caused an electron to jump from the donor to the acceptor over a distance of about four nanometers. The radical pair that formed from this reaction was extremely sensitive to weak magnetic interactions, proving that it is indeed possible for a radical-pair reaction to be influenced by the presence of—and, more important, the direction of—an Earth-strength magnetic field.
Schulten’s hypothesis also predicts that there must be sensory molecules (magnetoreceptors) in the retina in which magnetically sensitive radical pairs can be created using the wavelengths birds need for their compass to operate, which another line of research had identified as light centered in the blue region of the spectrum. In 2000 he suggested that the necessary photochemistry could take place in a then recently discovered protein called cryptochrome.
Cryptochromes are found in plants, insects, fish, birds and humans. They have a variety of functions, including light-dependent control of plant growth and regulation of circadian clocks. What makes them attractive as potential compass sensors is that they are the only known naturally occurring photoreceptors in any vertebrate that form radical pairs when they absorb blue light. Six types of cryptochromes have been found in the eyes of migratory birds, and no other type of candidate magnetoreceptor molecule has emerged in the past 20 years.
Like all other proteins, cryptochromes are composed of chains of amino acids folded up into complex three-dimensional structures. Buried deep in the center of many cryptochromes is a yellow molecule called flavin adenine dinucleotide (FAD) that, unlike the rest of the protein, absorbs blue light. Embedded among the 500 or so amino acids that make up a typical cryptochrome is a roughly linear chain of three or four tryptophan amino acids stretching from the FAD out to the surface of the protein. Immediately after the FAD absorbs a blue photon, an electron from the nearest tryptophan hops onto the flavin portion of the FAD. The first tryptophan then attracts an electron from the second tryptophan and so on. In this way, the tryptophan chain behaves like a molecular wire. The net result is a radical pair made of a negatively charged FAD radical in the center of the protein and, two nanometers away, a positively charged tryptophan radical at the surface of the protein.
In 2012 one of us (Hore), working with colleagues at Oxford, carried out experiments to test the suitability of cryptochrome as a magnetic sensor. The study used cryptochrome-1, a protein found in Arabidopsis thaliana, the plant in which cryptochromes had been discovered 20 years earlier. Using short laser pulses to produce radical pairs inside the purified proteins, we found that we could fine-tune their subsequent reactions by applying magnetic fields. This was all very encouraging, but, of course, plants don’t migrate.
We had to wait almost a decade before we could make similar measurements on a cryptochrome from a migratory bird. The first challenge was to decide which of the six bird cryptochromes to look at. We chose cryptochrome-4a (Cry4a), partly because it binds FAD much more strongly than do some of its siblings, and if there is no FAD in the protein, there will be no radical pairs and no magnetic sensitivity. Experiments in Oldenburg also showed that the levels of Cry4a in migratory birds are higher during the spring and autumn migratory seasons than they are during winter and summer when the birds do not migrate. Computer simulations performed by Ilia Solov’yov in Oldenburg showed that European Robin Cry4a has a chain of four tryptophans—one more than the Cry1 from Arabidopsis. Naturally, we wondered whether the extended chain had evolved to optimize magnetic sensing in migratory birds.
Our next challenge was to get large amounts of highly pure robin Cry4a. Jingjing Xu, a Ph.D. student in Mouritsen’s lab, solved it. After optimizing the experimental conditions, she was able to use bacterial cell cultures to produce samples of the protein with the FAD correctly bound. She also prepared versions of the protein in which each of the four tryptophans was replaced, one at a time, by a different amino acid so as to block electron hopping at each of the four positions along the chain. Working with these alternative versions of the protein would allow us to test whether the electrons are really jumping all the way along the tryptophan chain.
We shipped these samples—the first purified cryptochromes from any migratory animal—to Oxford, where Timmel and her husband, Stuart Mackenzie, studied them using the sensitive laser-based techniques they had developed specifically for that purpose. Their research groups found that both the third and fourth tryptophan radicals at the end of the chain are magnetically sensitive when paired with the FAD radical. We suspect that the tryptophans work cooperatively for efficient magnetic sensing, biochemical signaling and direction finding. We also speculate that the presence of the fourth tryptophan might enhance the initial steps of signal transduction, the process by which nerve impulses encoding the magnetic field direction are generated and ultimately sent along the optic nerve to the brain. We are currently conducting experiments to identify the proteins that interact with Cry4a.
One more cryptochrome finding deserves mention here. We compared robin Cry4a with the extremely similar Cry4a proteins from two nonmigratory birds, pigeons and chickens. The robin protein had the largest magnetic sensitivity, hinting that evolution might have optimized robin Cry4a for navigation.
Open Questions
Although these experiments confirm that Cry4a has some of the properties required of a magnetoreceptor, we are still a long way from proving how migratory birds perceive Earth’s magnetic field lines. One crucial next step is to determine whether radical pairs actually form in the eyes of migratory birds.
The most promising way to test for radical pairs inside the birds’ eyes was inspired by the work of chemists and physicists who, in the 1980s, showed that fluctuating magnetic fields alter the way radical-pair reactions respond to static magnetic fields. Their work predicted that a weak radio-frequency electromagnetic field, fluctuating with the same frequencies as the “singlet-triplet waltz,” might interfere with the birds’ ability to use their magnetic compass. Thorsten Ritz of the University of California, Irvine, and his colleagues were the first to confirm this prediction in 2004.
In 2007 Mouritsen began similar behavioral experiments in his lab in Oldenburg—with intriguingly different results. During the spring and fall, birds that travel between nesting and wintering grounds exhibit a behavior called Zugunruhe, or migratory restlessness, as if they are anxious to get on their way. When caged, these birds usually use their magnetic compass to instinctively orient themselves in the direction in which they would fly in the wild. Mouritsen found that European Robins tested in wooden huts on his university’s campus were unable to orient using their magnetic compass. He suspected that weak radio-frequency noise (sometimes called electrosmog) generated by electrical equipment in the nearby labs was interfering with the birds’ magnetic compass.
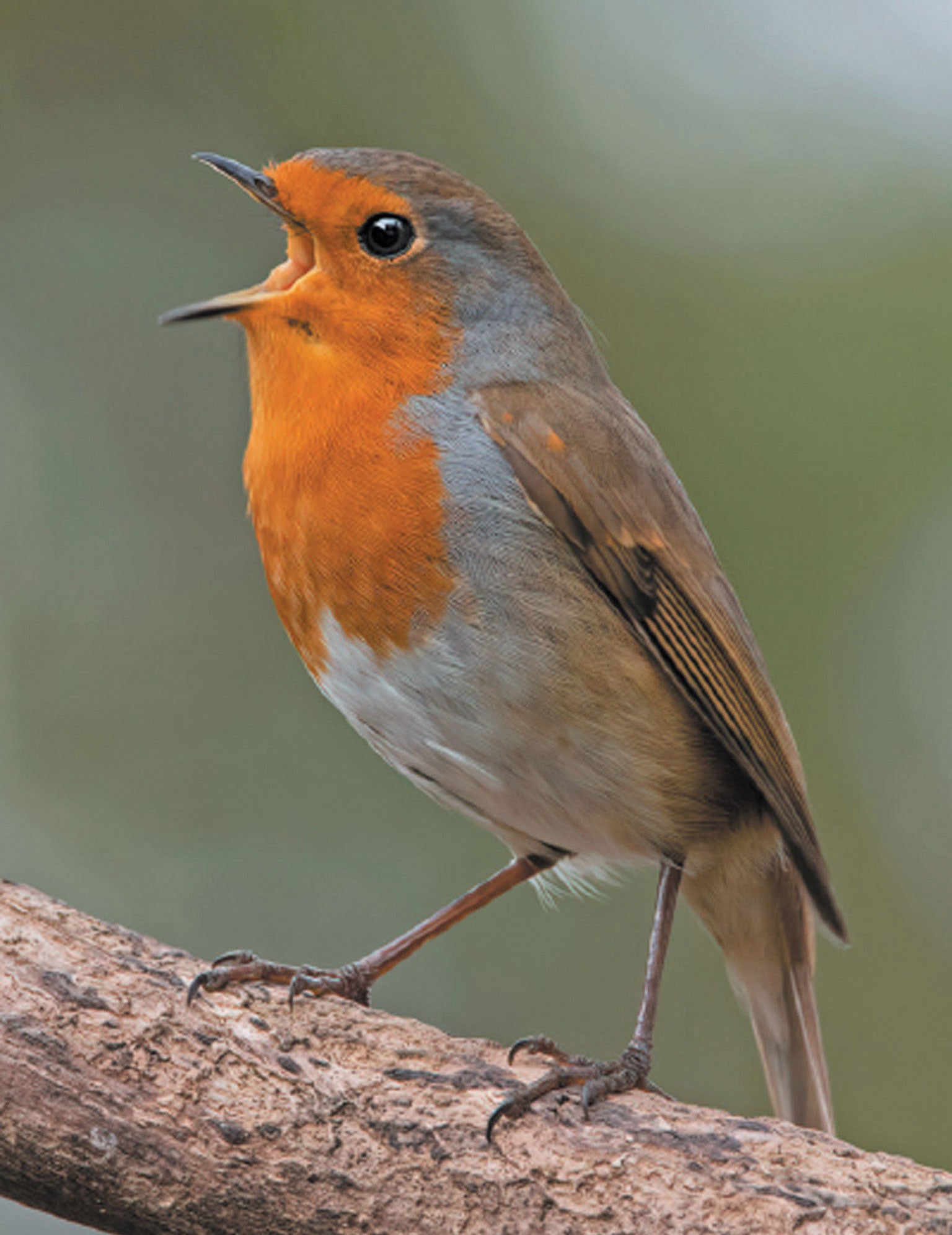
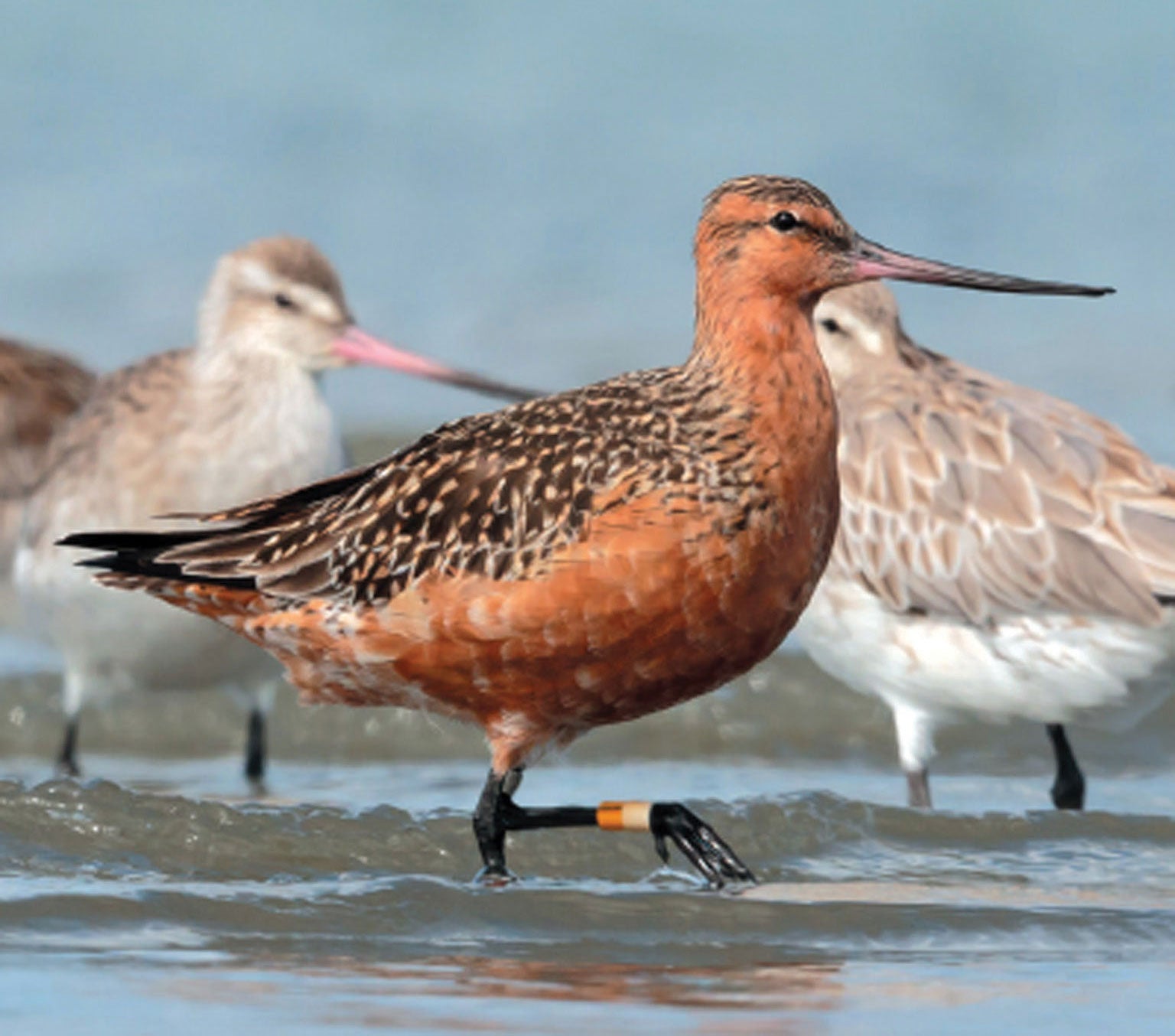
To confirm that electrosmog was the source of the problem, Mouritsen and his team lined the huts with aluminum sheets to block the stray radio frequencies. On nights when the shields were grounded and functioned properly, the birds oriented well in Earth’s magnetic field. On nights when the grounding was disconnected, the birds jumped in random directions. When tested in an unshielded wooden shelter typically used for horses some kilometers outside the city and well away from electrical equipment, the same birds had no trouble detecting the direction of the magnetic field.
These results are significant on several fronts. If the radio-frequency fields affect the magnetic sensor and not, say, some component of the signaling pathway that carries nerve impulses to the brain, then they provide compelling evidence that a radical-pair mechanism underpins the bird’s magnetic compass. The main competing hypothesis, for which there is currently much less support, proposes that magnetic iron–containing minerals are the sensors. Any such particles that were large enough to align like a compass needle in Earth’s magnetic field would be far too big to rotate in a much weaker field that reversed its direction millions of times per second. Furthermore, the radio-frequency fields that upset the birds’ magnetic orientation are astonishingly weak, and we don’t yet understand exactly how they could corrupt the directional information available from the much stronger magnetic field of Earth.
It is also remarkable that the birds in the Oldenburg lab were disoriented much more effectively by broadband radio-frequency noise (randomly fluctuating magnetic fields with a range of frequencies) than by the single-frequency fields mostly used by Ritz and his collaborators. We hope that by subjecting migratory songbirds to bands of radio-frequency noise with different frequencies we will be able to determine whether the sensors really are FAD-tryptophan radical pairs or whether, as some other investigators have suggested, another radical pair might be involved.
Many questions about the birds’ magnetic compass remain, including whether the magnetic field effects on robin Cry4a observed in vitro also exist in vivo. We also want to see whether migratory birds with genetically suppressed Cry4a production are prevented from orienting using their magnetic compass. If we can prove that a radical-pair mechanism is behind the magnetic sense in vivo, then we will have shown that a biological sensory system can respond to stimuli several million times weaker than previously thought possible. This insight would enhance our understanding of biological sensing and provide new ideas for artificial sensors.
Working to gain a full understanding of the inner navigation systems of migratory birds is not merely an intellectual pursuit. One consequence of the enormous distances migratory birds travel is that they face more acute threats to their survival than most species that breed and overwinter in the same place. It is more difficult to protect them from the harmful effects of human activity, habitat destruction and climate change. Relocating migratory individuals away from damaged habitats is rarely successful because the birds tend to instinctively return to those unlivable locales. We hope that by providing new and more mechanistic insights into the ways in which these extraordinary navigators find their way, conservationists will have a better chance of “tricking” migrants into believing that a safer location really is their new home.
When you next see a small songbird, pause for a moment to consider that it might recently have flown thousands of kilometers, navigating with great skill using a brain weighing no more than a gram. The fact that quantum spin dynamics may have played a crucial part in its journey only compounds the awe and wonder with which we should regard these extraordinary creatures.